Introductory Astronomy: Early 20th Century
Blackbody radiation is the
spectrum of light emitted by any opaque substance. An incandescent
light bulb is a good example: inside the bulb, a curly filament of
tungsten metal is heated by the passage of electricity until it
reaches a temperature of around 6000 Kelvin. Our eyes can see the
light (electromagnetic radiation) of a body this hot. A cooler
blackbody, like an electric stove burner, will look more red. A true
blackbody displays no spectral lines. In 1900, in searching for an
explanation for the spectral shape of the blackbody spectrum, Max
Planck found that he could predict the shape of the curve if he
assumed that light comes in little packets. Einstein, in 1905,
published a paper on the photoelectric effect that strongly
suggested that these little packets, or "quanta" (or
"photons" as we call them today) were real. Each photon
has an energy that is related to the wavelength that one would
measure with a spectrograph. This was the start of "quantum
mechanics." The word "quantum" simply means that many
physical quantities, like mass, light, and electricity come in
discrete packets: you cannot have 1.5 electrons, nor 3.2545 photons.
Also about the turn of the
century, J. J. Thompson isolated the electron, another very
important quantum.
Around 1911, Ernest Rutherford
discovered the basic structure of the atom: tiny, heavy, positively
charged nucleus at the center, surrounded by a vacuous cloud of
negatively charged, feather-light electrons.
The line spectrum of the hydrogen
atom was explained in 1913 by Neils Bohr. He assumed that, out of
the infinitely many orbits that the electron could have around the
proton, only a few would be allowed. These orbits he assumed were
circular and would not decay. The first orbit would have angular
momentum given by Planck's constant h, the second would have
angular momentum 2h, the third 3h and so on. The
electron could occupy one of these orbits at a time. If the electron
jumps up or down a level, a photon is either released or absorbed.
If excited to a high orbit, electrons drop down levels on their own,
over time, releasing photons. The energy differences between levels
correspond to the photon energies of the released photons. Since
only a few energy levels exist, this explains why an emission
spectrum have only a few lines at very precisely-defined wavelengths
- those are the wavelengths that correspond to the orbit changes.
The excitation of an electron to a higher orbit can be accomplished
by (1) absorption of a photon of the correct energy, or (2)
collision with an electron or ion.
Other elements are not so easy to
deal with mathematically as Hydrogen (which is composed of only two
particles, an electron and a proton), but each element's spectral
pattern is unique.
Radioactivity: Early work was
done by A. H. Becquerel, Pierre, and Marie Curie. Uranium and newly
discovered Polonium and Radium were found to be radioactive, meaning
that they emitted particles that had not been seen before, termed
alpha-, beta-, and gamma-rays by the Curies. Gamma-rays turned out
to be another kind of light, with ultra-short wavelengths and very
large photon energies. Beta-rays turned out to be electrons, and
alpha-rays turned out to be the nuclei of Helium atoms, that
is, two protons and two neutrons stuck together and ejected from the
radioactive substance without opportunity to attract 2 electrons to
balance the protons' charge.
More on Ernest Rutherford: in
1919, building on a student's work, Rutherford bombarded nitrogen
gas with alpha particles from a radioactive source. Stunningly, the
nitrogen transformed into oxygen plus a proton (Hydrogen atom). This
was the first observed nuclear reaction, which tend to be
millions of times more energetic than ordinary chemical reactions
such as combustion because they involve nuclei rather than the
fluffy outmost electrons.
James Chadwick discovered the
neutron in 1932, a particle without charge almost exactly the same
mass as a proton. Quickly, a new picture of the nucleus solidified.
Neutrons are needed in every nucleus (except H) to hold the nucleus
together against the enormous repulsion force of the protons. This
force is now called the strong nuclear force (the weak nuclear force
was already established from radioactivity).
E=mc2 is a statement
(from Einstein's theory of special relativity) that matter can be
transformed into energy and that energy can be transformed into
matter. This comes into play with nuclear reactions. For instance,
when 4 Hydrogen fuse into one He nucleus, as in the sun, the 4 H
nuclei weigh more than the He nucleus by about 0.7 percent. The mass
that "disappeared" became energy (photon energy plus heat
plus the ejection of a few neutrinos).
Lise Meitner was forced to flee her native Austria in 1938
by the rise of Nazi Germany, and, in Sweden, discovered a
peculiarity in the behavior of Uranium that was to rock the world.
She was able to figure out the following equation, where a rare
isotope of Uranium is bombarded by neutrons: 92U235
+ 0n1 = 56Barium141 +
36Krypton92 + 30n1,
where the top number is charge and the bottom number is mass of the
nucleus. Because of the extra neutrons coming from the split Uranium
nucleus, this sets up a "chain reaction" in which the
neutrons start more reactions which yield more neutrons which start
more reactions and so on. This uncontrolled chain reaction was
utilized in world war II in the two atomic bombs dropped on
Hiroshima and Nagasaki.
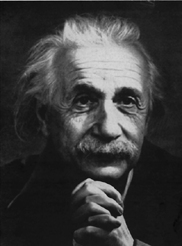
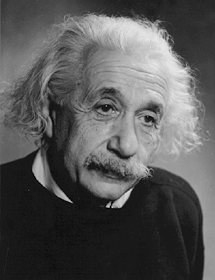
Alfred Einstein: In summary, there are 3 main things
Einstein did that are directly relevant to this course. (1) He
solidified the notion that light quanta (photons) are real with his
1905 paper on the photoelectric effect. (2) Also in 1905, he
published the "special theory of relativity" in which
different observers moving at different speeds experience the
passage of time at different rates (there is no such thing as
absolute time). The famous formula E=mc2 that represents
the rest energy of any bit of matter comes from this theory. (3) By
1915, Einstein had worked out the "general theory of
relativity" which extended special relativity into accelerated
frames of reference - that is, gravity fields. This theory of
gravity replaces Newton's! It predicts (a) the bending of starlight
near a gravitating body - gravitational lensing, (b) deviations from
Newton's gravity law in strong gravity fields - this explains a
long-standing anomaly in the rate of precession of Mercury's orbit,
(c) gravitational radiation, and (d) provides the rules for the
geometry of the universe.
Last
modified: Thu Oct 19 08:21:50 CDT 2000